Basic Choices and Constraints on Long−Term Energy Supplies
Population
growth and energy demand are exhausting the world's fossil energy
supplies, some on the timescale of a single human lifespan.
Increasingly, sharing natural resources will require close
international cooperation, peace, and security.
Human society, like any system composed of dynamic processes, depends on
an external energy source. Historically, that source was the Sun, which
provides heat, light, and photosynthesis for food to support work
energy by man and animal, and affects wind and water motion. Since the
early 19th century, though, the discovery of and access to a vast
supply of fossil fuels within Earth has enabled the industrial
revolution, near−exponential growth of population,1 technologies, and wealth. That period could well be renamed the energy revolution (see figure 1).
As we enter a new millennium, we are growing increasingly concerned
about the limits of our fossil fuels that are driving the world's
economies. Many journal articles, committee reports, and books have
addressed this "energy problem"; they contain opinions, ideas, and
suggestions from experts within their various subdisciplines on
possible ways to improve our practices or innovate technologically. But
a complex interdependence exists among the technological, social, and
environmental aspects of energy use (see the articles in Physics Today, April 2002).
Furthermore, many of the ideas researchers propose cannot significantly
impact the real magnitude of the energy problem or may provide only
short−term relief. Our basic choices are limited. Nature's energy
resources are confined to two categories: Earth−stored fossil residues
and nuclear isotopes, whose economic utility is limited by the finite
amounts that exist on Earth, and the radiation flux of solar energy,
whose economic utility is limited by the finite rate at which we can
capture the Sun's energy and by the land areas that societies can
dedicate to harness it. The longevity of the fossil energy supply and the
net rate of solar energy availability are both reduced by the energy
consumed through their conversion to a suitable energy form and the
technologies that accompany that conversion: storage, delivery,
maintenance, and repair of environmental damage. Solar−derived consumer
energy, whether as electricity, biomass, or wind, represents a clean,
alternative energy form. It is important to understand a basic law of
nature: Energy, once used, is not regenerable. So the public term
"renewable energy" is misleading. The following analysis examines the magnitudes of
the world's energy supplies and the basic constraints on our ability to
support in the long term society's demands using those finite supplies.
To put those magnitudes into a human context for policymakers and the
public, the longevity of our resources will be expressed on the scale
of a human lifespan (where 1 human lifespan is approximately 75 years).
Energy demands
In viewing overall societal energy issues, it is useful to express energy magnitudes in units of the quad (Q), where 1 Q = 1015 BTU, roughly equal to 2.5 × 1014 kcal or 1.06 × 1018 joule. Current US energy consumption is about 100 Q/year, roughly a quarter of the world's total demand.2
Energy demand by humanity continues to rise. An increase of about 1.5%
per year is projected in the US and world demand is expected to
increase by 1−2% per year for many decades, mainly due to continued
population growth. While total demand is, of course, influenced by
personal demand, even unusually large (20%, say) conservation efforts
would be nullified by population growth in less than 20 years.
Earth−stored resources
-
Petroleum. In 1956, petroleum geologist M. King Hubbert correctly
predicted that a peak and subsequent drop in US production would occur
around 1970.3 In fact, foreign imports
have since risen to 60% of current consumption. US dependence on
foreign petroleum is certain to increase.
In
2000, Jay Hakes of the Energy Information Administration presented a
similar and extensive US Department of Energy assessment of the likely
trend and peak in the world petroleum supply.4 Figure 2
shows the predicted range of years when the peak is likely to occur for
demand whose growth rate may be between 0−2%. Because growth rates due
to population alone are anticipated to be at least 1% per year for many
decades to come, the pivotal event is expected to occur well within a
human lifespan. Moreover, the analysis was based on an optimistic
estimate of the world oil resource of approximately 2200−3900 billion
barrels, nearly twice the proven reserve.5 That would place the anticipated time to reach the peak well within a few decades.
- Natural gas. A natural gas shortage exists now in the US. Yet the current growth rate of US demand is approaching 3% per year.2,6 As seen in figure 3, the proven US natural gas reserve would last very few years, even at constant (year 2000) demand.
Estimated
gas reserves worldwide are relatively large. Geologists have good
reasons to believe the sum of our reserves and still undiscovered (but
likely to exist) natural gas could last roughly for another 45−60 years
(see figure 3).2,6
However, those reserves are widely scattered around the world: 58% are
reported to be located in Russia, Iran, and Qatar, with small
contributions in numerous other countries.2
Clearly, their use will depend on vast international and
intercontinental transportation by pipelines, transoceanic shipment as
liquefied natural gas (LNG), or advance conversion to liquid fuels.
Energy sacrifice by basic thermodynamic requirements plus process
efficiency loss will accompany advance conversion to liquid fuels.
Geopolitical cooperation will be essential.
- Coal.
The largest fossil fuel resource available in the US is coal. The energy
content of the current US reserve is about 5667 Q. If demand remains
frozen at the current rate of consumption, the coal reserve will indeed
last roughly 250 years.2 That prediction assumes equal use of
all grades of coal, from anthracite to lignite. Population growth alone
reduces the calculated lifetime to some 90−120 years (see figure 4).
Any new uses of coal would further reduce the supply. The
Fischer−Tropsch process has been used to convert coal to gasoline motor
fuel in South Africa for decades, for example. The process requires
that one carbon atom of coal be sacrificed to generate at least two
hydrogen atoms, and it takes energy to decompose water to make that
hydrogen. As a result, the process consumes 2 Q of coal to generate 1 Q
of motor fuel. Hydrogen production would require an even greater
consumption of coal. The use of coal for conversion to other fuels
would quickly reduce the lifetime of the US coal base to less than a
human lifespan (see figure 4).
High carbon dioxide emissions also accompany the conversion of coal to any motor fuel. For more details on how CO2 complicates the energy problem, see the box on page 50.
- Dilute fossil residues.
Oil shale, or bitumen, is sedimentary rock containing dilute amounts of
"heavy oil" or near−solid carbonaceous residues. The US has negligible
amounts of that resource. Worldwide estimates of the total energy
contents are large but highly speculative.2,7
To harvest the dilute solid carbonaceous contents requires drastic
measures: Either underground combustion, heating, steam, or air to
drive the carbonaceous solids toward the surface, or the mining of huge
volumes of solids using heat, solvents, and steam to extract the
resource. The extracts must be further processed to yield usable
hydrocarbon fuels, a process that requires further energy sacrifices.
Compared to petroleum, these heavy oils present additional refining and
environmental problems because of the abundance of nitrogen, oxygen,
and metal compounds found in them. Also, the amount of CO2 released during processing and use greatly exceeds that released by the current use of petroleum fuels.
-
Nuclear energy. Uranium fission plants in the US are presently
supplying less than 8% of our total energy demand. Were the current
nuclear technology expanded to provide the electricity now supplied by
coal (about 23 Q), the estimated US uranium resources2 would be exhausted in about 35−58 years—less than a human lifespan.
Constraints on solar energy use
The amount of solar energy received across US latitudes is approximately 22 Q per year per 4000 km2 (about a million acres) on average.8
Technologies based on this resource have the potential to become major
contributors to our energy supplies (see Sam Baldwin's article in Physics Today, April 2002, page 62.)
Photovoltaic solar cells convert 10−20% of incident radiation directly
to electricity. Figure 5 illustrates how large a surface area of cells
would be required to generate a particular amount of electricity. The
yellow region indicates electricity produced directly at the cell. The
blue region is a more realistic mapping and indicates the larger cell
areas needed to cover the energy losses in transformers, transmission,
power−equalization over time, and efficiency losses that occur for any
conversion to gaseous or liquid fuels. Thus about 40−80 thousand km2
of area—roughly 2−4 times the size of Massachusetts—could supply about
20 Q, or 20−25%, of today's US total energy requirements. That amount and more of available land can
probably be found in the US. But the size illustrates the magnitude of
the technological and social impact. It is instructive to compare what
fraction of other nations' total areas would be required to supply
their current energy demand. The percentage ranges from as low as 0.2%
for Australia to as much as 24% of the land occupied by Belgium (see the table on page 51). The data assume a 15% solar−cell efficiency, and 50% efficiency at the site of consumption.
Biomass energy production requires photosynthesis exclusively on fertile
land, but it is another much discussed alternative energy. The US has
about 1.6 million km2
(400 million acres) of arable land that provides food for the current US
population, with about 20% of the food left for export. The US is
likely to progressively need that 20% in the next few decades as its
population increases. Moreover, the current agricultural productivity
depends on fossil fuels to provide the reactive nitrogen required to
make fertilizer. Otherwise, about three to four times that 1.6 million
km2 of arable land will be needed to provide photosynthetic nitrogen fixation to generate the current food supplies.
Quite apart from fertile land requirements, the solar−to−biomass
conversion efficiency is very much smaller than for the conversion of
solar to electrical energy. Modern agriculture can generate about 1−1.5
million kg of biomass vegetation per square kilometer of land with
about 16 000 BTU per kg, for a total of about 0.06−0.09 Q on 4000 km2
of land. However, after accounting for external energy consumed through
the agricultural process and the conversion of biomass to a useful
fuel, the net energy production, if any, is less than 0.02 Q on 4000 km2—two
orders of magnitude smaller than that of photovoltaic cell conversion.
That is, biomass conversion would require some 100−fold more area of
fertile land. Wind energy is another secondary product of solar
radiation. Although few studies have assessed its ultimate
technological promise, researchers estimate that the technology could
potentially generate a maximum of 3−22 Q of electricity in the US.9
Energy losses due to transmission, supply, and demand fluctuation or
conversion to other energies will reduce the actual contribution, but
wind energy provides a significant potential resource contribution.
Hydrogen fuel from solar−cell electricity would be free of CO2 emissions, but the "hydrogen economy" would depend on vast land areas as illustrated by the yellow band in figure 5.
In addition to energy losses during conversion to hydrogen, energy
losses will occur in the creation and operation of a vast new
infrastructure designed to store, ship, distribute, and handle huge
amounts of hydrogen at all levels, from manufacture to uses. In a
recent analysis, Reuel Shinnar10 of the
City College of New York noted that the enormous effort to alter our
infrastructure to create a hydrogen economy argues strongly for the
direct automotive use of electricity itself, for which much of the
infrastructure and potential technology are at hand.
Energy science
Energy availability determines, drives, limits, and shapes the working capability of all processes of society.11
The silent and plentiful gift of energy has fundamentally influenced the
application of economic theory as well as the teachings of most other
disciplines in the educational system.
- Economics. In the 1970s, Nicholas Georgescu−Roegen12
tried to demonstrate the actual relationship between economics and
thermodynamics, the basic physics of energy. He observed that most
economists believe that "the economic process can go on, even grow,
without being continuously fed low entropy," which in a thermodynamics
context means "without receiving new energy." As we approach the limits
of our easy access to energy, the defining economic currency will be
dominated by availability of energy units rather than by an artificial
currency, be that gold or dollars.
This change in
economic theory is well illustrated by the silicon photovoltaic cells
that brilliantly accomplished their mission in space flight in 1972 at
an affordable economic cost. Yet, if they had to provide us with
indispensable alternative energy, they would have had to operate
continuously for at least 20 years just to replace the energy invested
(or consumed) in their production. By 1999, photovoltaic cells were
reported to produce their investment energy in about 3−7 years.13
That history illustrates the profound economic importance of the concept
of net energy. The economic value of an alternative energy technology
depends on the net rate of energy QNE it will deliver after the rate of energy production QPR is debited by the energy consumed for its operation QOP and the energy invested in its creation E during its lifetime T:
QNE = QPR − (QOP + E/T).
For example, ethanol production from biomass, which involves a complex
agricultural and industrial processing system that requires large and
diverse external energy inputs QOP, easily results in a negative QNE, yet government subsidies can make the production profitable to producers.
- Education. The
educational system has become focused on how to manage, produce,
distribute, and enjoy the objects, services, and pleasures that
plentiful energy makes possible. That system has grown into ever more
disciplines and subdisciplines that serve ever more specialized skills.
Dedication to basic science—that is, to the laws of nature that allow,
control, and constrain all abilities and potentials—is no longer
emphasized. Basic science remains limited largely to recitation of
formalisms that are gladly forgotten after examination time because
little effort is made to relate their basic and universal relevance to
specialties, the totality of life, and society.
More than ever since the beginning of the energy revolution, knowledge
of the basic nature and limits of energy is needed to realistically
determine and carry out effective policy designed to guarantee reliable
energies in the future. That could well help ensure the survival of
civilization. As H. G. Wells once remarked, "Human history more and
more becomes a race between education and catastrophe."
A knife−edge issue
The major source of the world's energy supply, the fossil fuels, will
decline in availability within several decades. It is of paramount
importance that the public and policymakers recognize the ensuing
shortages and the urgent need for policies that will address them. In
particular, an urgent commitment to solar and nuclear energy
technologies appears to be mandatory for the long term. Solar energy technology offers the most promising
capabilities for the future because photovoltaic cells can generate
potentially large quantities of electricity for nations with sufficient
land area. Worldwide use, though, will depend on international peace
and cooperation.
Current uranium fission technologies could provide enough energy for a few decades.14 Advanced fission technologies that involve breeder methodologies and the use of thorium, as envisioned by Edward Teller,15
could extend that timeline to many hundreds of years. Controlled nuclear
fusion remains a unique energy alternative of vast magnitude. Moreover,
nuclear technologies are not dependent on location and land area. At
the moment, public concern over potential risks has virtually stopped
the pursuit of this energy source. Peaceful cooperation among nations will be
increasingly and vitally important for accessing and sharing our
remaining resources. Human society faces no greater risk, however, than
ignorance of the basic laws of nature, the role and finite magnitudes
of energy sources, the arithmetic of population growth (see Albert A.
Bartlett's article in this issue on page 53),
and their consequences on the survival of humanity. As Shirley Ann
Jackson, president of the American Association for the Advancement of
Science, points out (see APS News, October 2003, page 8), "The
public policy arena needs the voice of science itself . . . weighing in
on knife−edge issues with the voice of reason."
I acknowledge the tireless assistance of David Pimentel, professor
of agricultural sciences at Cornell University, for advice on
agricultural science; its role in food production, land use, and
biomass production; and their relevance to energy issues.
Paul B. Weisz is
an emeritus professor of chemical and bioengineering at the University
of Pennsylvania and a retired senior scientist and manager at the
Central Research Laboratory of the Mobil Corp. He is also currently an
adjunct professor of chemical engineering at the Pennsylvania State
University.
3. M. K. Hubbert, in Drilling and Production Practice, American Petroleum Institute, Washington, DC (1956); M. K. Hubbert, Am. Assoc. Pet. Geol. Bull. 51, 2207 (1967).
6. National Petroleum Council Committee on Natural Gas, Natural Gas: Meeting the Challenges of the Nation's Growing Natural Gas Demand, vol. 1, National Petroleum Council, Washington, DC (1999).
7. W. Youngquist, Shale Oil: The Elusive Energy, newsletter no. 98/4, M. King Hubbert Center for Petroleum Supply Studies, Golden, CO (1998).
8. W. E. Reifsnyder, H. W. Lull, Radiant Energy in Relation to Forests, US Dept. of Agriculture, Forest Service, Washington, DC (1965); E. P. Odum, Fundamentals of Ecology, 3rd ed., W. B. Saunders, Philadelphia (1971); M. Slesser, C. Lewis, Biological Energy Resources, Wiley, New York (1979); S. B. Weiss, Can. J. Forest Res. 30, 1953 (2000).
9. D. Pimental et al., BioScience 52, 1111 (2002); EIA Monthly Energy Review, DOE/EIA−0035(95/02), Washington, DC (February 1995).
10. R. Shinnar, Technol. in Soc. 25, 455 (2002).
11. P. B. Weisz, in Chemical Engineering in a Changing World, W. T. Koetsier, ed., Elsevier Scientific, New York (1976).
12. N. Georgescu−Roegen, The Entropy Law and the Economic Process, Harvard U. Press, Cambridge, MA (1971).
13. R. Corkish, Sol. Prog. 18, 16 (1997).
15. E. Teller, Memoirs: A Twentieth−Century Journey in Science and Politics, Perseus, Cambridge, MA (2001), p. 565.
17. M. M. Maroto−Valer et al., Am. Chem. Soc. Div. Fuel Chem. 49, 373 (2004).
18. K. L. Griffin, J. R. Seemann, Chem. Biol. 3, 245 (1996) [MEDLINE]; H. Elderfield, Science 296, 1618 (2002) [MEDLINE].
Figure 1. World population growth since the 13th century.
Return to Article
Figure 2. Predictions indicate that the peak and
subsequent decline in world oil production will probably occur within
the next few decades. The data here are based on optimistic estimates
that place the oil reserve at 2248−3896 billion barrels. Just how soon
the peak will occur depends on annual population growth rates and
increases in demand. The given ranges account for uncertainty in
predicting the future: For each estimate of projected growth in demand
for petroleum—0, 1%, or 2%—there exists a 95% chance that the peak will
occur by the year on the left−hand end of the range and a 5% chance
that it may occur as late as the year on the right−hand end. (Data from ref. 4.)
Return to Article
Figure 3. Outlook for world and US natural gas
capacity, based on current proven gas reserves and on currently
estimated resources (including unproven and nonproduceable amounts).
The energy content is given to the right of each bar, the length of
which indicates the amount of time that the natural gas supply is
likely to last. That longevity depends on the annual growth in usage
(shown on the far right) that may occur over the next few decades.
(Based on data from ref. 6, and ref. 2, Energy Information Administration International Energy Outlook 2004.)
Return to Article
Figure 4. Outlook for the longevity of the US coal
supply, based on the current consumption rate and a range of
anticipated annual growth rates—up to a 2% increase in demand per year.
The two lowest bars indicate the longevity of the coal supply if coal
is converted to other fuels. Experts estimate that roughly 54% of the
reserve underground—comprising anthracite, bituminous, subbituminous,
and lignite rock—is recoverable. (Data from ref. 2, Annual Energy Review 1999.)
Return to Article
Box1: Basic Problems Associated with Carbon Dioxide Emissions
The massive quantities of carbon dioxide currently generated during
fossil fuel consumption are responsible for progressive global warming.
This problem has become a matter of global concern and has led to large
efforts and expenditures for research in technologies designed to
sequester CO2.16
Unfortunately, permanent immobilization confronts fundamental problems. Like H2O, CO2
is a chemically inert molecule. Its only potential reaction partners
possibly available in sufficient magnitudes may be mineral oxides—for
example, calcium− and magnesium−silicates. They exist in dense
geological formations. However, no useful reaction rate is achievable
in such locations. Their use would require mining, shipping, grinding,
special activation processing,17 and disposal of gigatons of the solids.
Most prominent research projects are directed toward massive physical storage of CO2
by injection into those geological formations or within the deep oceans
(see Jorge L. Sarmiento and Nicolas Gruber's article in Physics Today, August 2002, page 30). It is difficult to accurately predict the integrity of such physical storage over long periods16
because many variables in complex environments are involved. Attempts to
manipulate marine or terrestrial ecosystems and increase the amounts of
CO2 these sinks naturally hold are fraught with great complexities that involve multiple and interactive processes.18
Any conversion of a carbonaceous fossil fuel to a fuel of lower carbon
content—including the conversion all the way to hydrogen—will eject the
excess carbon as CO2.
The problem of its emission to the atmosphere is simply transferred
from the points of consumption to the location where the conversion
process takes place. Therefore, the CO2 problem is not
eliminated by a "hydrogen economy" if the hydrogen is created by the
conversion of coal, petroleum, or natural gas.
Return to Article
Figure 5. Photovoltaic cell areas required to generate
electrical energy that could supply a sizable fraction of the US
economy. The yellow area plots the electrical energy produced at the
solar cell surface for efficiencies between 10 and 20%. The blue area
plots the energy at the consumer side and accounts for losses in
transmission, storage, and so forth, in addition to efficiency losses.
Some US states (Delaware, Massachusetts, Indiana, Idaho, Arkansas, and
California) and world nations provide the scale of the enormous land
areas that would be required.
Return to Article
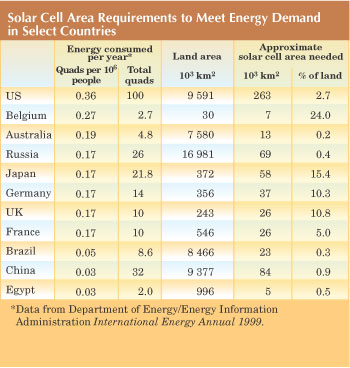
Return to Article
© 2004 American Institute of Physics
|
|
Sponsored links
|